The Utility and Practice of EDX Testing in the Pediatric Population: An AANEM Consensus Statement
Peter B. Kang MD 1,2 | Hugh J. McMillan MD, MSc3 | Nancy L. Kuntz MD4 |
Tanya J. Lehky MD5 | Katharine E. Alter MD6 | Kevin F. Fitzpatrick MD7 |
Charbel El Kosseifi MD8 | Susana Quijano-Roy MD8 |
Abstract
Nerve conduction studies and needle electromyography, collectively known as electrodiagnostic (EDX) studies, have been available for pediatric patients for decades, but the accessibility of this diagnostic modality and the approach to testing vary significantly depending on the physician and institution. The maturation of molecular diagnostic approaches and other diagnostic technologies such as neuromuscular ultrasound indicate that an analysis of current needs and practices for EDX studies in the pediatric population is warranted. The American Association of Neuromuscular & Electrodiagnostic Medicine convened a consensus panel to perform literature searches, share collective experiences, and develop a consensus statement. The panel found that electrodiagnostic studies continue to have high utility for the diagnosis of numerous childhood neuromuscular disorders, and that standardized approaches along with the use of high-quality reference values are important to maximize the diagnostic yield of these tests in infants, children, and adolescents.
K E Y W O R D S
adolescents, children, electrodiagnostic medicine, electromyography, infants, neuromuscular disorders, pediatric
- Division of Pediatric Neurology, Department of Pediatrics, University of Florida College of Medicine, Gainesville, Florida
- Department of Neurology, University of Florida College of Medicine, Gainesville, Florida
- Department of Pediatrics, University of Ottawa and Children's Hospital of Eastern Ontario, Ottawa, Ontario, Canada
- Department of Pediatrics, Northwestern University Feinberg School of Medicine and Lurie Children's Hospital, Chicago, Illinois
- National Institute of Neurological Disorders and Stroke, Bethesda, Maryland
- Functional and Applied Biomechanics Section, Rehabilitation Medicine, National Institutes of Health Clinical Center, Bethesda, Maryland
- Inova Neuroscience and Spine Institute, Inova Fairfax Hospital, Falls Church, Virginia
- Centre de Référence Maladies Neuromusculaires, Service de Neurologie, Réanimation et Réeducation Pédiatriques, Hôpital Raymond Poincaré, Garches, France
This research was supported in part by the Intramural Research Program at the National Institute of Neurological Disorders and Stroke, National Institutes of Health
Abbreviations: AANEM, American Association of Neuromuscular & Electrodiagnostic Medicine; CCM, corneal confocal microscopy; CMAP, compound muscle action potential; CMS, congenital myasthenic syndrome; EDX, electrodiagnostic; EMG, electromyography; LEMS, Lambert-Eaton myasthenic syndrome; MCD, mean consecutive difference; MEP, motor evoked potential; MUAP, motor unit action potential; NCS, nerve conduction study; RNS, repetitive nerve stimulation; SFEMG, single-fiber electromyography; SMA, spinal muscular atrophy; SPACE, stimulation potential analysis with concentric electrodes; SSEP, somatosensory evoked potential; tcMEP, transcranial motor evoked potential.
1. INTRODUCTION
Electrodiagnostic (EDX) testing has been used to evaluate children with suspected neuromuscular disorders for decades. With the widespread adoption of genetic testing in the 1990s and 2000s, questions arose regarding whether the utility of EDX testing in the pediatric population would decline to obsolescence.1 On the contrary, EDX tests have continued to show utility for the diagnostic evaluation of children, and patient referrals for these studies have remained at least stable overall, and have even been documented to be increasing in some centers.2 The mix of referral questions has changed, however; fewer patients with suspected muscular dystrophy are being referred for EDX testing, while evaluations for traumatic and other acquired injuries have increased.2 Additionally, selected standard electrophysiologic measures, such as compound muscle action potentials (CMAPs), are being used in pediatric clinical trials, along with specialized electrophysiologic measures such as motor unit number estimation.3 Despite this stable and perhaps increasing demand for pediatric EDX testing, the availability of these tests varies from center to center, along with the approaches to performing the studies. For these reasons, the American Association of Neuromuscular & Electrodiagnostic Medicine (AANEM) convened an expert panel to review the literature and discuss their collective experiences to provide some guidance to performing these diagnostic tests in the pediatric population.
2. METHODS
Literature searches were performed on PubMed (www.ncbi.nlm.nih. gov/pubmed) for keywords relevant to the following subjects: (1) indications for EDX testing; (2) sedation and consent issues, parental presence during needle electromyography (EMG); (3) technical issues including stimulator size; (4) normative data including late responses; (5) specialized nerve conduction studies (NCSs), including repetitive stimulation and blink reflexes; (6) needle EMG issues; (7) single-fiber EMG (SFEMG); (8) small nerve fiber testing; and (9) evoked potentials. The literature searches included the keywords “pediatric” or “children,” or alternatively were restricted to an age range of 0-18 years. Details of individual literature searches for the individual topics may be found in Supporting Information Supplemental Methods, which is available online. The retrieved abstracts were screened manually by individual panel members and relevant articles identified for detailed analysis. Whenever possible, articles focusing primarily on the pediatric population were used. Review articles and single case reports were generally excluded. Panel members also contributed previously known articles relevant to these topics in cases where the literature search did not yield any relevant articles, or to supplement the results of the literature searches. The selected articles were then analyzed for contributory data; these data are summarized and interpreted below.
3. RESULTS
3.1 | Indications for EDX testing
The indications for ordering EDX testing in children have evolved over the decades. In 1983, Bady et al. published a series of 1624 studies in 1385 children performed over a 3-year period.4 Within a subset of 122 children with hypotonia and weakness, 48% of studies were abnormal, and the most common diagnoses were spinal muscular atrophy (SMA), congenital myopathy, and peripheral neuropathy. In 2000, Darras and Jones published a review that addressed this issue, and recommended that EDX studies be ordered as an early diagnostic study when the diagnoses of congenital myopathy, indeterminate proximal weakness, inflammatory neuropathy, inherited neuropathy, and disorders of the neuromuscular junction were under consideration.1 They recommended that EDX studies be used as a second tier test for other diagnoses such as facioscapulohumeral muscular dystrophy, myotonic dystrophy, periodic paralysis, and SMA. They noted that at a major pediatric referral center, the volume of studies hovered in the 100-150 range annually from 1979 to 1999, and noted no perceptible decrease in patient volume, despite the growing availability of diagnostic genetic testing during that period.
A follow-up article from the same center in 2014 noted that EDX testing volume hovered around 150 cases per year from 2001 to 2007, then increased significantly to 200-250 annually from 2008 to 2011, suggesting that EDX testing continues to be useful, despite ongoing advances in genetic testing.2 That study found that common indications for ordering an EDX study in children include evaluations for suspected polyneuropathy, mononeuropathy, and various focal neurological symptoms in one or more extremities.
Mononeuropathies are less common in the pediatric population than in adults, yet they constitute a significant percentage of nerve injuries in this age group, and EDX studies play a critical role in the diagnosis and monitoring of such injuries. This was recognized at least as far back as the 1980s.5
In the upper limb, carpal tunnel syndrome, the most common mononeuropathy in adults, is relatively rare in children, but this disease does occur, often in the setting of mucopolysaccharidosis,6,7 congenital bone abnormalities in the wrist,8 as a manifestation of hereditary neuropathy with liability to pressure palsies,9,10 or as an isolated hereditary disease,7,9,10 and less commonly in the context of Schwartz-Jampel syndrome.9 Proximal median neuropathies comprise a more significant proportion of median neuropathies in children compared with adults.11 Ulnar neuropathy, the most common upper limb mononeuropathy in childhood, is associated with both axonal and demyelinating physiology,12 and is often caused by trauma, compression, and entrapment.12,13 The final major upper limb mononeuropathy, radial neuropathy, has similar common etiologies of trauma, compression, and entrapment, generally with a good prognosis.14 In contrast to adults, radial neuropathy in childhood does not typically localize to the spiral groove; the posterior interosseous nerve and distal main radial trunk are more common sites of injury.15
In the lower limbs, sciatic neuropathy in childhood has been associated with trauma,16 iatrogenic causes,16 compression,16 various tumors,16,17 including perineurioma,18 and sometimes has vascular causes,16 including occlusion of the inferior gluteal artery.19 The other major mononeuropathy in the lower limbs, fibular (peroneal) neuropathy, has been associated with compression, trauma, and entrapment.20 One cause of compression of the fibular nerve is osteochondroma at or near the fibular head.21 As in adults, fibular neuropathy in children is frequently associated with axonal and fascicular injury.22 Clubfoot has on occasion been associated with EDX abnormalities, most commonly fibular neuropathy.23,24
There is ongoing debate in the literature regarding the utility of EDX studies in the setting of neonatal brachial plexus palsy. Some surgeons argue that EDX data do not add meaningfully to information obtained by means of history and physical examination, or may underestimate the severity of some lesions.25 However, a survey of peripheral nerve surgeons found that a majority request EDX studies before surgical intervention.26 Many surgeons and EDX physicians would likely agree that EDX studies play a role in the evaluation of neonatal brachial plexus palsy, but that EDX data should be placed in the context of the entire clinical evaluation and not interpreted in isolation.27
EDX studies have been found to be useful in the evaluation of outcomes in pediatric facial reanimation procedures.28
3.2 | Special considerations, including consent and optimization of patient comfort
Neurophysiologic studies of the motor unit produce valuable information to guide diagnosis and treatment planning. However, the approach to performing studies in infants, children, and adolescents needs to be individualized to the age and developmental stage of the patient to obtain maximal data. Most of the “art” of pediatric EDX testing has been shared through mentoring and common experiences; however, well-written summaries discuss methods and technique.29,30
It is important for the physician to understand methods of obtaining informed consent for EDX testing. One practice has the referring physician discuss the clinical need (potential benefits) of the testing while deferring a more detailed discussion of the actual test process to the physician performing the study. This clinician can describe the proposed testing (potential risks) using optimum vocabulary: “electrical pulses” rather than “shocks,” “wire probes” rather than “needles.” While there was individual variability, a formal prospective study of pediatric patients at Great Ormond Street Hospital in London demonstrated that children over the age of 4 years objectively graded discomfort from needle EMG in the same range as that of venipuncture.31
Minimizing pain and discomfort for the patient is of critical importance in pediatric EDX testing. Protocols for EDX testing in awake children are individualized to the diagnostic question with consideration for the child's developmental/emotional state. In most cases, starting with the appropriate sensory NCSs would be optimal: sensory nerves frequently require lower levels of stimulation and pure sensory nerve stimulation does not produce a muscle twitch. Longer intervals between each individual nerve stimulus (even when building toward supramaximal stimulus) help children tolerate NCSs. In small children, toddlers, and infants, stimulation above 50 mA is rarely needed. Increases in stimulus intensity from one stimulation to the next should be limited to approximately 5 mA.32 Minimizing the number of supramaximal stimulations is also helpful. The full complement of nerve testing is accomplished more quickly while administering each stimulus at longer intervals in children, with fewer premature refusals to continue. Completion of the EMG before allowing the child to examine the needle electrode is usually helpful.
A survey of EDX practitioners in 1992 identified younger children, children who had been uncooperative with previous painful procedures and those whose mothers reported fear and anxiety about undergoing EDX testing as factors predicting behavioral distress during EDX tests.33 Avoiding unneeded anxiety-provoking explanations, providing support from Child Life professionals and appropriately supportive parents, and providing pleasant distraction in terms of music/ videos/playful interaction all help many children tolerate the procedures without requiring pharmacologic interventions.
Distraction (ideally with the assistance of a Child Life specialist) has been found to be effective at reducing pain and should be used whenever practical. Several pharmacologic options also exist to accomplish this goal.29 Topical anesthetic preparations, subcutaneous lidocaine injections, vapocoolant sprays, and needle-free lidocaine jet injection systems have been used at various centers. Another alternative pain reduction method is a commercially available device that combines an ice pack with mechanical vibration. This device has been reported to reduce pain from needle insertions including intramuscular injections and other procedures.34 It is widely used by pediatricians for immunizations and venipuncture in children. This device is used by some pediatric EDX physicians for EMG electrode insertions as well as for other injections, including botulinum toxin (K.E.A., personal communication).
The degree of distress or pain tolerated by infants and children for elective medical tests in an outpatient setting varies throughout the world; thus, it is critically important to match appropriate patients with the ideal pain-minimizing procedures. In addition, the ages between 18 months and 5 years are frequently ones when noncompliant behavior is at its peak. In consideration of the above, conscious sedation and/or general anesthesia are used in some centers to facilitate neurophysiologic testing at these ages. While many anesthetic agents depress central activity and reflexes (eg, electroencephalographic background rhythms, cortical evoked potentials, blink reflexes, H reflexes, and F responses), they do not impair peripheral motor and sensory nerve conduction. Deeper levels of anesthesia clearly inhibit voluntary movement; however, use of combinations such as ketamine and midazolam or nitrous oxide and midazolam allow children to move intermittently or in response to reflex activation allowing analysis of motor unit recruitment and characteristics. Tetanic stimulation at 10 Hz can be used to simulate exercise needed during testing of neuromuscular transmission.
3.3 | Technical issues
A variety of technical factors impact the performance and interpretation of pediatric NCS and EMG. It is important to appreciate these concepts to provide appropriate interpretation of data obtained and arrive at a correct diagnosis.
Standard, adult nerve stimulators generally have a distance of 3 cm between the anode and cathode. These are generally acceptable for patients above the age of 18-24 months. In younger patients, stimulators with shorter distances between anode and cathode (as little as 0.5 cm) are preferable.32,35 For NCSs performed on children, it is also preferable to use a stimulating device that allows the examiner's hand to encircle the limb being studied while simultaneously holding the stimulating electrode. Small, bar-style stimulating electrodes are ideal for this purpose.30
Due to the shorter limb lengths in pediatric patients as compared to adults, it is not always possible or preferable to use stimulator-torecording electrode distances that have been standardized for adult patients. For these reasons, it is often necessary to use anatomic landmarks, and not standardized distances, to determine stimulation sites.32 For the same reason, recording-to-reference electrode distance (which is standardized to at least 3 cm in adult sensory NCSs) may need to be modified for pediatric patients. Thus, adult bar-style recording electrodes with fixed interelectrode distances are not practical for some pediatric NCSs.
Standard-sized reusable electrodes are insufficient for recording over very small surfaces, as is often required in pediatric NCSs. Most disposable, stick-on electrodes can be cut to allow for smaller recording surfaces, making them a necessity for pediatric NCSs.35
To minimize pain and discomfort during needle electrode examination, the smallest possible needle should be used. Concentric, 30-gauge (0.30 mm diameter), 1-inch-long EMG needles (sometimes referred to as “facial” needles) should be adequate for most needle electrode examinations in pediatric patients, although it is important that the length of the needle be adequate to pass through subcutaneous fat, which may be more prominent in children, especially in infants and toddlers.30 Furthermore, minimizing the number of muscles to be studied is critical to the success of a pediatric EMG. In various institutions, some studies are completed with needle electrode examination of only a single muscle based on considerations of expected additional diagnostic data to be obtained with further study weighed against the goal of maximizing patient comfort.30 Of course, the number of muscles to be tested is dependent on the suspected diagnosis and clinical presentation of the patient, but should be minimized in all pediatric tests.
3.4 | Normative data including late responses
Normative data for NCSs from infancy to adolescence has become increasingly available in recent years. Interpreting NCS data for young children must take age into consideration as axon diameter and myelination gradually increase from birth until adult values are attained at 4-5 years of age.36,37 Whereas a healthy adult is predicted to have an ulnar motor nerve conduction velocity > 52 m/s,38 ulnar motor nerve conduction velocities for a healthy full-term newborn are expected to be 20-36 m/s36,39 and a premature infant at 25 weeks gestational age would be expected to have velocities <15 m/s.40 Correspondingly, serial NCSs for preterm infants show gradual increases in conduction velocities toward term values during the third trimester.40
Neurophysiologists have established ranges of normal values for sensory and motor amplitudes, distal latencies, and conduction velocities for children. Awareness of the physiological changes that occur during infancy and early childhood is essential to avoid misinterpretation of normal NCSs. The AANEM's Normative Data Taskforce established criteria for evaluating articles under consideration as normative standards.14 Applying those criteria to published pediatric studies yielded seven articles published in peer-reviewed journals since 1980 that provide high-quality normative data, from preterm infants42 to older children43-48 (Table 1). One additional article with a cohort of 92 children was included as it approached the 100-patient threshold established by the AANEM task force49 (Table 1).
The oldest study in the modern era was published by Lang et al.48 Of particular interest is this study's compilation of data separated by sex. Nerve conduction velocities tended to be higher in girls compared with boys, but statistical analyses were not conducted for those differences. Parano et al.47 provided normative data on 155 children segregated by various age groups. Cai and Zhang45 included a large cohort of 168 children, the majority of whom (N = 85) were less than 1 year old. This study, therefore, provides useful data for the incremental increases in conduction velocity and amplitude that occur with the physiological increase in axon diameter (thereby increasing conduction velocity) and in the case of motor studies, physiological muscle fiber hypertrophy (reflected in increased CMAP amplitudes) in early childhood. Garcia et al.49 provided values for 92 children aged 1 week (born full-term) to 6 years. Wang et al.44 is unique in that it provides data for femoral sensory and motor NCSs in 163 healthy children, including F-wave latencies and detection rates for proximal F-wave responses (see below). Although femoral NCSs are not routinely performed in most children, they may nevertheless be useful for those with quadriceps weakness and/or suspected femoral neuropathy.
The most recent study, with by far the largest cohort, is Ryan et al.,43 which includes data from a large set of 1,918 NCSs performed on 1,849 unique pediatric patients, stratified into multiple age groups. Lower limits for amplitude and conduction velocity, and upper limits for distal latency, were provided for values at the 5th percentile or 95th percentile, respectively (ie, 2 SD below or above the mean). In light of the comprehensive nature of the data and the extensive set of tables, this article provides high-quality data that may be used in pediatric EDX laboratories on a daily basis. A limitation of this study was the low sample size for infants. Data from this single study should be compared with those from other studies as needed, especially for infants.
Smit et al.42 was the only large study of 200 preterm infants aged 25-30 weeks gestational age. Motor nerve conduction velocities were provided for median and tibial nerves with normative data provided including thresholds of 2 SD below the mean for each week of gestational age achieved. The gradual improvement of conduction velocity in this preterm cohort is similar to that reported in a smaller study.40
Advances in statistical and computational resources now allow neurophysiologists to calculate reference ranges for individual EDX laboratories given an adequate amount of data from those laboratories. The analyses from one such approach are referred to as enorms.50 It is important to ensure that robust sample sizes are used (>100 patients) and that developmental factors are considered (physiologically small axon diameters and incomplete myelination for children less than 5 years) to place these calculations into the proper developmental context.
Height should be taken into account for children as nerve conduction velocity is inversely proportional to an individual's height.48 This may also be an important consideration for late responses (H-reflex) performed in rapidly growing adolescents.
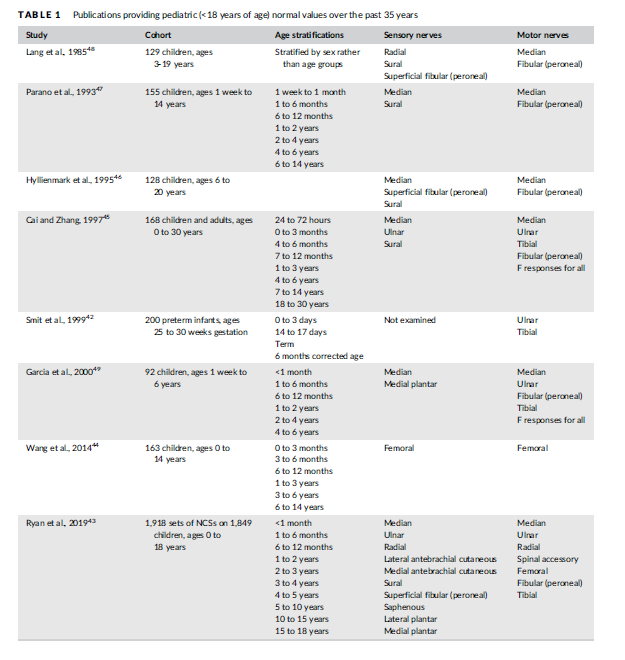
Subcutaneous fat, particularly in younger children (<2 years old), can pose technical challenges and can make it difficult to elicit sural or superficial peroneal sensory nerve responses consistently in this age group. Medial and lateral plantar responses may be measured orthodromically in such younger children to overcome this challenge.
As in adults, effects of temperature must be carefully considered in the pediatric age group. In normal subjects, within a range that excludes very high or very low temperatures, a 1C decrease in local temperature results in a 1.2 to 2.4 m/s decrease in motor and sensory conduction velocities.51 Infants may be more susceptible to cooling (due to their large surface area) and overly anxious children may have cooler limbs due to the autonomic nervous system shunting blood away from extremities. Standardization of temperature during testing is necessary to obviate this potential artifact. Upper limb temperatures should be maintained at 34-36C and lower limbs at 32-34C).
Eight articles pertaining to normal pediatric F response latencies were found from the past 35 years (Table 2). Five of the studies also provided data pertaining to sensory and motor nerve conductions studies as noted above,44,45,47,52,53 whereas three studies focused specifically on F-wave data for children <18 years old.39,54,55 Six studies provided infant data,39,44,45,47,54,55 of which five reported a progressive decrease in minimum F-response latency from the neonatal period (< 28 days), early infancy (1-6 months), older infancy (7-12 months), to the toddler years (1-3 years).39,44,45,54,55 This age-related change is due to progressive increases in axon diameter and myelination. Only one study reported an increase in F-response latency from 1 week to 7 years of age.47 For the six studies that compared minimum F-wave latencies form infancy throughout childhood, all reported a steady increase in F-wave latency from the toddler years (1-3 years old) to school entry47,54,55 and into adolescence,44,45,52 attributable to linear growth. Height is the most significant factor influencing the minimum F response latencies, more so than age, suggesting that height should be accounted for when interpreting F response latencies, especially in rapidly growing adolescents.52
With respect to other late responses, H-reflex reference values in children are available from a cohort of 91 children (ages 1 day to 12 months).56 Normal values have also been calculated for a novel Treflex measuring the latency of myoelectric deep tendon (T) reflex response in children, recorded from the soleus and rectus femoris.57
3.5 | Specialized NCSs: Repetitive stimulation and blink reflexes
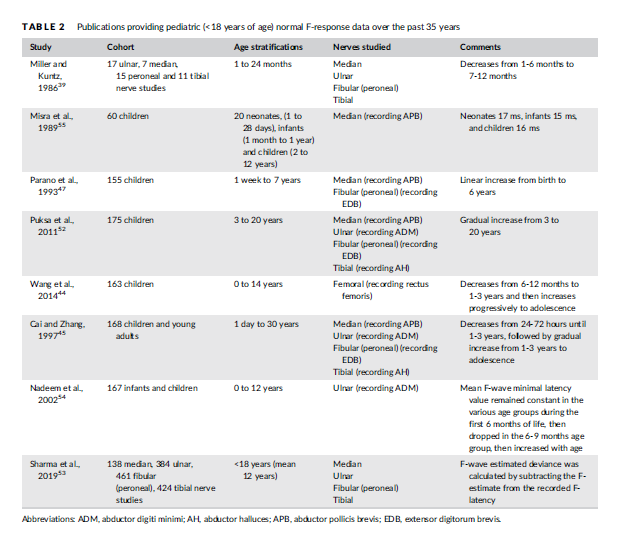
Repetitive nerve stimulation (RNS) and exercise testing can provide useful information in the evaluation of a child with a suspected disorder of neuromuscular transmission. RNS can help facilitate the diagnosis of disorders such as transient neonatal myasthenia gravis or congenital myasthenic syndrome (CMS). Many infants and children with disorders of neuromuscular transmission may present with weakness and breathing or feeding difficulty, which can show clinical and electrophysiological overlap with myopathies,58,59 including several inherited neuromuscular diseases.60 As such, a careful interrogation of the function of the neuromuscular junction is an important consideration. RNS is commonly used after routine NCSs have been completed, but it is important to note that, as in adults, important information can be obtained from routine motor studies. Routine motor NCSs may elicit “double” CMAP responses, which can be a clue to the presence of certain types of CMS, including slow-channel CMS or acetylcholinesterase deficiency.61 While this can be a helpful clue, the sensitivity of this electrophysiological finding is not known. Moreover, it is not specific to CMS as it can also be seen with organophosphate exposure.62 A failure of neuromuscular transmission may lead to reduced CMAP amplitudes as well.
Slow RNS (2 to 3 Hz) in children is performed in a similar manner as in adults. Supramaximal stimulation is delivered as a train of 5 or 10 pulses. The CMAP amplitudes of the first and fourth (or fifth) responses are compared, and a CMAP decrement of >10% is considered abnormal. While this test can provide important information, it is uncomfortable and can be difficult for young children or those with cognitive impairment to tolerate and cooperate. At least 30-65% of children with juvenile myasthenia gravis may initially present with ocular symptoms,63,64 and the likelihood of a child presenting with ocular (rather than generalized) myasthenia gravis symptoms is reportedly higher in prepubertal (vs post pubertal) children.65 This must be considered because RNS of the facial nerve (stimulating anterior to the tragus and recording from the nasalis or orbicularis oculi) is particularly challenging for younger children and less likely to be performed successfully without sedation or general anesthesia. Adult studies have found SFEMG to be more sensitive than RNS at diagnosing a disorder of neuromuscular transmission.66,67 Although these data are lacking in children, many pediatric neurologists and neurophysiologists would concur that SFEMG or even stimulated SFEMG is a more sensitive tool.68 SFEMG may also detect abnormalities in clinically unaffected forearm muscles in adult patients presenting with ocular forms of the disease.69
Transient neonatal myasthenia gravis is commonly diagnosed with RNS because this tends to be a more generalized disorder as a result of the passive transfer of maternal antibodies.70
Fast RNS (20 to 50 Hz) is needed to test for possible facilitation or increment in CMAP amplitude in presynaptic disorders of neuromuscular transmission such as infantile botulism, presynaptic CMS71,72 and pediatric Lambert-Eaton myasthenic syndrome (LEMS). There are case reports of infants with CMS (including choline acetyltransferase deficiency caused by CHAT mutations) who have subsequently been found to have abnormalities on fast or prolonged RNS.71,73 Although these diagnoses are individually rare, all have potential clinical implications if overlooked, including apnea (botulism or CHAT mutations) or paraneoplastic causes (LEMS).74
Exercise testing has diagnostic value for patients with suspected muscle channelopathies as it may document abnormal membrane excitability. Standardized protocols comprising the short and long exercise tests have been developed to differentiate among these disorders during neurophysiological testing and different patterns of abnormality can provide evidence for a potential sodium or chloride channelopathy.75 Limb cooling may also help to differentiate the two.76 Of note, these tests require cooperation on the part of the patient and are, therefore, more likely to be of value in older children and adolescents.
Facial nerve motor NCSs, blink reflex studies, and facial muscle EMG have roles in evaluating infants and children with facial or bulbar weakness. They may assist with localizing the site of injury, which can include: muscle (eg, nemaline rod myopathy), peripheral nerve (eg, birth trauma), or brainstem nuclei (eg, brainstem dysgenesis). Normal values for facial nerve motor NCSs are available for age ranges throughout childhood.77
Blink reflex testing evaluates integrity of the trigeminal and facial nerves as well as the pathways between their cranial nuclei. A supramaximal shock is applied to the supraorbital branch of the trigeminal nerve resulting in a direct response that has two components: R1 (rapid and brief) and R2 (delayed and prolonged). The R1 response is present from birth and achieves normal adult values (range, 9-13 ms) at 4 weeks of life for a term infant.78 The ipsilateral R2 (R2i) can be elicited in most term newborns (latency range, 34-43 ms); however, the contralateral R2 (R2c) is not consistently obtained before the age of 9 months old.79 The initial absence of an R2c is thought to be due to lack of normal myelination of the brainstem, facial, and trigeminal pathways in very young children.80 Latency differences between R2i and R2c have also been reported in normal infants <12 months old, with physiological differences in latencies lessening after that age, so that the latencies are identical to adults by 3 years old.81
Interpretation of blink reflex findings in children is otherwise similar to that of adults. Abnormality of the blink reflex has been reported as an early electrophysiological sign of acute inflammatory demyelinating polyneuropathy82 as well as in children with myelomeningocele,83 children with Tourette syndrome,84 or adolescents and adults with migraine,80 although practically it is not used for any of these conditions.
While electrophysiological testing can reliably elicit R1 and R2i responses from birth, observational studies have noted that administering a shock to the glabellum reliably elicits a blink response in 100% of term infants,85 whereas handheld esthiometers elicited a blink in only 25% of infants at 1 week old, slowly increasing to 100% of infants by 12 weeks of age.86 An intact blink reflex has been consistently shown to be a good predictor of clinical outcome for idiopathic facial palsy (Bell's palsy) in adults87,88 as well as in children.89
Blink reflex is abnormal in children with Moebius syndrome and other disorders of brainstem dysgenesis, a finding that can aid in the diagnosis of these conditions.90,91
For infants with facial or bulbar dysfunction and concern regarding potential feeding difficulty, simultaneous needle EMG of the genioglossus and thyrohyoid have been used at specialized centers to permit electrophysiological evaluation of infants clinically suspected to have dyscoordinated sucking and swallowing.77
3.6 | Needle EMG issues
EMG is generally a necessary and valuable complement to NCSs.2,92 In studies addressing an isolated traumatic nerve injury, the focus of the needle EMG study is directed by clinical examination of weakness and likely nerve(s) involved. In diagnostic studies that assess generalized neuromuscular disorders, the choice and number of muscles to study is more complicated. The aim is to optimize the diagnostic value of the study while minimizing discomfort to the child. The sensitivity of EMG in neurogenic diagnoses can be as high as 99.5%.93 Myogenic disorders are less sensitive to diagnosis by EMG in ages under 2 years old,1,93-95 although sensitivity improves in the older age groups.
Review of the literature suggests that EMG of at least one arm and leg is recommended when feasible, with four muscles being optimal for making a diagnosis.94 This allows for sampling muscles in a wide anatomic distribution but may be limited by patient cooperation; thus, in many cases the clinical context and the results of the NCSs indicate that sampling one muscle is most prudent, as noted above. Pediatric studies looking at pain perception equate the EMG with venipuncture.31 There is increased perception of pain when more than one muscle is sampled and in the upper extremities (including genioglossus in this study). Another consideration is the effect of using smaller pediatric concentric needles instead of standard concentric needles, which may lower motor unit action potential (MUAP) amplitude without a significant effect on MUAP duration.96 With these considerations, it is judicious to initially choose the most appropriate muscle to sample.
Neurological exam cannot be overemphasized as well as knowledge of the suspected type of disorder. Muscle ultrasound before needle EMG can scan several muscles quickly and is painless.97 Using ultrasound to identify abnormal-appearing muscles and to estimate the severity of abnormalities increases the likelihood of choosing muscles for needle EMG that will display clear diagnostic findings. Sedation in performing EMG studies is used to varying degrees in different institutions, some places perform sedated procedures routinely94 and others more rarely.1 Sedation has the advantage of permitting the study to be performed without upsetting the child. The limitation is a reduced ability to volitionally activate many muscles to evaluate motor unit activity. The risk of anesthesia in a child also needs to be considered, particularly if there is severe pulmonary or cardiac involvement.98
One representative EDX laboratory familiar to two of the authors (T.J.L. and K.E.A.) is the EMG Laboratory at the National Institutes of Health, where both awake and sedated pediatric studies are performed. In complicated situations where multiple diagnostic tests are needed, sedated studies are usually performed in a multidisciplinary facility that also includes specialized personnel for MRI imaging, audiology, dental, ophthalmology, cerebrospinal fluid studies (lumbar puncture), and skin biopsy. In sedated studies and in addition to standard NCSs, these two authors sample two muscles in the leg (tibialis anterior and internal hamstrings) and one muscle in the arm (biceps). These muscles are chosen because they are easiest to activate in a lightly sedated patient while others, muscles particularly in the hand, are more difficult to activate. Other studies such as RNS or SFEMG may be performed if clinically indicated. In awake patients, the EDX physician is more dependent on clinical and ultrasound examination to choose the muscle most likely to be abnormal for needle EMG. For cooperative children, more than one muscle is examined.
3.7 | Jitter analysis for disorders of the neuromuscular junction: SFEMG and stimulation potential analysis with concentric electrodes
Analysis of jitter is the most sensitive study of the neuromuscular junction.99,100 This is an important diagnostic study for CMS, botulism, and neonatal myasthenia gravis. In the pediatric population, traditional stimulated SFEMG techniques have been used.73 In addition, an adaptation of the stimulated SFEMG technique, using a concentric needle electrode to record from the orbicularis oculi, has been used successfully by several groups. This adapted technique is known variously as SPACE (stimulation potential analysis with concentric electrodes),101-103 stimulated jitter analysis,100,104 or concentric needle jitter.105 The zygomatic branch of the facial nerve is stimulated by a monopolar needle. In SPACE and stimulated jitter studies, a concentric facial needle is used to collect a sufficient number of single motor fiber unit potentials. The advantage of these adapted techniques is that they can be performed sedated or with local anesthesia and within approximately 15-20 min. The disadvantage is that there may be biasing of the potentials leading to a decreased jitter.106 Normative values in the pediatric population are limited by the ability to perform studies on normal children. Using an analytic technique of e-norms, it was determined that children over the age 2 years have jitter values (mean consecutive difference [MCD], 22 μs) approaching adult levels (MCD, 21 μs).50 Children younger than 2 years old have slightly longer jitter values; with 1- to 2-year-olds having an MCD of 33 μs, and those under 1 year having an MCD of 45 μs.107
The diagnostic value of jitter in the orbicularis oculi varies in the different CMS.59 Patients with DOK7 CMS are less likely to have an abnormal jitter. Also, the possibility of a neurogenic disorder needs to be ruled out because it can cause a false positive result.
3.8 | Small nerve fiber testing
Small nerve fibers are thinly myelinated A delta and unmyelinated C fibers that mediate the sensations of pain and temperature and autonomic function. Autonomic symptoms that can be produced by dysfunction of autonomic small nerve fibers include: dry eyes, dry mouth, orthostatic symptoms (from lightheadedness through syncope), palpitations, variations in blood pressure, abnormal sweating, overheating, erectile dysfunction, nausea, vomiting, constipation, early satiety, urinary frequency, nocturia and others.
Evaluation of small fiber function is important in children as there are several disorders associated with dysfunction limited to these fiber types in which standard NCSs are unrevealing. These include erythromelalgia,108 Fabry disease,109 diffuse widespread pain,110-112 and familial dysautonomia, and other hereditary neuropathies.113,114
Sympathetic skin response testing is a simple technique that can be performed using standard EDX equipment. Surface electrodes are placed over the palm and sole of the feet and can record sympathetic responses to a variety of stimuli: tactile, auditory, or electrical pulses.115 However, this response habituates quickly to repeated stimuli and the response is poorly graded and so usually assessed qualitatively as present or absent. Standard motor and sensory NCSs do not evaluate small nerve fibers.
Patient-reported outcome surveys have been developed and validated for adults with small fiber polyneuropathies.116 These surveys have not been validated in children or adolescents; however, they have been used informally with perceived benefit (Oaklander A.L., personal communication).
Computer-assisted quantitative sensory testing can be used to assess temperature and pain perception and has demonstrated reproducible results in children as young as 6 years of age.117 Values of cold sensation, warm sensation, cold pain, heat pain and vibration sensation detection thresholds were determined in the hand and foot for healthy children between 6 and 17 years of age.
Intraepidermal nerve fiber measurements have been used to quantify the status of small nerve fibers in humans.118 The invasiveness of performing one to several 3-mm full-thickness skin punch biopsies and the absence of normative values has limited use of this technique in children. However, recent modifications to technique (obtaining suction blister specimens on extremities) have been correlated to values measured on full-thickness skin punch biopsies and quantified in 20 healthy children between 7 and 17 years of age.119
Corneal confocal microscopy (CCM) is a noninvasive method for measuring small nerve fiber branching, density, and length. CCM can be scored in an automated manner and does not require undue degrees of cooperation so would appear to be a promising technique for quantifying small nerve fiber function in children.120
Contact heat evoked potentials require a heated foil thermode to apply cycles of heating and cooling on an extremity while the evoked potential is recovered over a CZ scalp electrode. The technique does not cause tissue injury and is considered noninvasive. There are no published reports using this technique in children.121
Measurements of the extent of surface area involved and the volume of sweat generated by standard heat stresses have been used with several techniques (thermoregulatory sweat testing and quantitative sudomotor axon reflex testing) to evaluate small fiber function.122,123 These techniques have been used and demonstrated to contribute significantly to diagnosis and treatment planning in various disorders in children and adolescents.123
Other autonomic tests evaluating vagal, sympathetic, and gastrointestinal function have been demonstrated to be useful while contributing to diagnosis, prognosis, and treatment planning.123
In conclusion, evaluating small nerve fiber function is important for all who care for children and adolescents. However, the techniques for this testing reach beyond standard NCSs and needle EMG. Techniques such as thermoregulatory sweat testing can be used at present with good validity and interpretability. However, validation of reproducibility and knowledge of age appropriate normal values for younger children do not yet exist for other commonly used autonomic tests, such as the Valsalva ratio and heart rate response to deep breathing,
3.9 | Evoked potentials
There is an extensive literature on the use of evoked potentials in children. However, the bulk of the articles focus on audiologic, ophthalmologic, or central nervous system applications of this test modality, which are not the focus of the current discussion. With respect to lower motor neuron / peripheral nervous system applications, somatosensory evoked potentials (SSEPs) and motor evoked potentials (MEPs) were historically used for diagnostic purposes,124 but those applications have become less common with the wide availability of spine MRI technology and other diagnostic tests. Currently, evoked potentials frequently have utility in children as intraoperative monitoring modalities during procedures such as spine surgeries.124 Intraoperative neurophysiologic monitoring using SSEPs, MEPs, and/or EMG (ideally all three simultaneously) is useful whenever nervous system tracts are at risk during operative procedures, so that any potential damage to such tissues may be prevented or mitigated. MEPs are often generated using transcranial electrical stimulation, yielding transcranial MEPs, also known as tcMEPs. Technical details of tcMEPs in children such as the optimal number of pulses during spine surgeries have been studied.125 The interactions between MEPs and various anesthetic agents have been studied, and MEPs are preserved in children who are administered either propofol or desflurane.126 In contrast, dexmedetomidine tends to attenuate tcMEP signals.127
4 | DISCUSSION
Literature review revealed that a significant proportion of the literature on the use of EDX testing in pediatrics consists of articles published before 2000; however, it is notable that there continues to be a steady stream of papers that continue to the current day on this topic. This reflects the stable and even increasing volume of children who undergo these studies and demonstrates the continued utility of EDX testing in children, albeit with a shift in the proportions of various indications and diagnoses. Most notably, certain categories of inherited diseases such as muscular dystrophy and SMA do not routinely require EMG as part of the diagnostic evaluation. However, in atypical cases EDX testing can provide critical assistance with narrowing of the differential diagnosis.
Data on normative values are difficult to gather in large quantities, especially for children. It is heartening to see that seven articles met rigorous criteria to help determine what normative values to use on a routine basis. It is also encouraging to see that one of these articles was published recently, in 2019.
It is important for any medical center that provides care for a significant number of children to provide this service to patients who would benefit from it, performed by trained EDX physicians who should have a strong background in pediatrics.
The panel concludes that the use of EDX testing in children will thrive for some time to come, and that techniques and practice patterns for this important diagnostic test modality will continue to evolve in the future. EDX testing in children will continue to complement other diagnostic test modalities such as various serum tests, muscle biopsy, imaging, and genetic testing.
ACKNOWLEDGMENTS
The authors thank the AANEM and Millie Suk, JD, for their support for this project.
CONFLICT OF INTEREST
P.B.K. reports consulting for AveXis (Novartis) and ChromaDex, advisory board activities for Sarepta Therapeutics, and honoraria from Springer (co-editor for textbook on pediatric electromyography), Wiley (for editorial work on Muscle & Nerve), and Wolters Kluwer (authorship for topics in UpToDate). H.J.M. reports consulting for Avexis (Novartis) and has received honoraria from Springer (co-editor for textbook on pediatric electromyography). N.L.K. reports advisory board service for Audentes Therapeutics, AveXis (Novartis), Biogen, Cytokinetics, Roche, and Sarepta Therapeutics. T.J.L., K.E.A., K.F.F., and C.E. report no disclosures. S.Q.-R. reports advisory board activities for AveXis (Novartis), Biogen, and Roche.
ETHICAL PUBLICATION STATEMENT
The authors confirm that they have read Muscle & Nerve's position on issues involved in ethical publication and affirm that this report is consistent with those guidelines. This article underwent review by the AANEM Professional Practice Committee and by the Editor-in-Chief, but did not undergo external peer review.
SUPPORTING INFORMATION
Additional supporting information may be found online in the Supporting Information section at the end of this article.
References
- Darras BT, Jones HR. Diagnosis of pediatric neuromuscular disorders in the era of DNA analysis. Pediatr Neurol. 2000;23(4):289-300.
- Karakis I, Liew W, Darras BT, Jones HR, Kang PB. Referral and diagnostic trends in pediatric electromyography in the molecular era.
- Muscle Nerve. 2014;50(2):244-249.
- Kang PB, Gooch CL, McDermott MP, et al. The motor neuron response to SMN1 deficiency in spinal muscular atrophy. Muscle Nerve. 2014;49(5):636-644. Bady B, Vila A, Boulliat G, et al. Value of electromyography in the child. Apropos of 1,624 examinations performed over a 3 year period. Rev Electroencephalogr Neurophysiol Clin. 1983;13(3):282-288.
- Jones HR Jr. Compressive neuropathy in childhood: a report of 14 cases. Muscle Nerve. 1986;9(8):720-723.
- Gschwind C, Tonkin MA. Carpal tunnel syndrome in children with mucopolysaccharidosis and related disorders. J Hand Surg Am. 1992; 17(1):44-47.
- Van Meir N, De Smet L. Carpal tunnel syndrome in children. J Pediatr Orthop B. 2005;14(1):42-45.
- Potulska-Chromik A, Lipowska M, Gawel M, Ryniewicz B, Maj E, Kostera-Pruszczyk A. Carpal tunnel syndrome in children. J Child Neurol. 2014;29(2):227-231.
- Cruz Martinez A, Arpa J. Carpal tunnel syndrome in childhood: study of 6 cases. Electroencephalogr Clin Neurophysiol. 1998;109(4): 304-308.
- Davis L, Vedanarayanan VV. Carpal tunnel syndrome in children.
- Pediatr Neurol. 2014;50(1):57-59. Deymeer F, Jones HR Jr. Pediatric median mononeuropathies: a clinical and electromyographic study. Muscle Nerve. 1994;17(7): 755-762.
- Karakis I, Liew W, Fournier HS, Jones HR Jr, Darras BT, Kang PB. Electrophysiologic features of ulnar neuropathy in childhood and adolescence. Clin Neurophysiol. 2017;128(5):751-755.
- Felice KJ, Royden Jones H Jr. Pediatric ulnar mononeuropathy: report of 21 electromyography-documented cases and review of the literature. J Child Neurol. 1996;11(2):116-120.
- Escolar DM, Jones HR Jr. Pediatric radial mononeuropathies: a clinical and electromyographic study of sixteen children with review of the literature. Muscle Nerve. 1996;19(7):876-883.
- Karakis I, Georghiou S, Jones HR, Darras BT, Kang PB. Electrophysiologic features of radial neuropathy in childhood and adolescence. Pediatr Neurol. 2018;81:14-18.
- Srinivasan J, Ryan MM, Escolar DM, Darras B, Jones HR. Pediatric sciatic neuropathies: a 30-year prospective study. Neurology. 2011; 76(11):976-980.
- McMillan HJ, Srinivasan J, Darras BT, et al. Pediatric sciatic neuropathy associated with neoplasms. Muscle Nerve. 2011;43(2):183-188.
- Ferraresi S, Garozzo D, Bianchini E, Gasparotti R. Perineurioma of the sciatic nerve: a possible cause of idiopathic foot drop in children: report of 4 cases. J Neurosurg Pediatr. 2010;6(5):506-510.
- Srinivasan J, Escolar D, Ryan M, Darras B, Jones HR. Pediatric sciatic neuropathies due to unusual vascular causes. J Child Neurol. 2008;23(7):738-741.
- Jones HR Jr, Felice KJ, Gross PT. Pediatric peroneal mononeuropathy: a clinical and electromyographic study. Muscle Nerve. 1993;16(11): 1167-1173.
- Levin KH, Wilbourn AJ, Jones HR Jr. Childhood peroneal neuropathy from bone tumors. Pediatr Neurol. 1991;7(4):308-309.
- Karakis I, Khoshnoodi M, Liew W, et al. Electrophysiologic features of fibular neuropathy in childhood and adolescence. Muscle Nerve. 2017;55(5):693-697.
- Song KS, Kang CH, Min BW, Bae GC, Cho CH, Lee JH. Congenital clubfoot with concomitant peroneal nerve palsy in children. J Pediatr Orthop B. 2008;17(2):85-89.
- Thometz J, Sathoff L, Liu XC, Jacobson R, Tassone JC. Electromyography nerve conduction velocity evaluation of children with clubfeet. Am J Orthop (Belle Mead NJ). 2011;40(2):84-86.
- Abid A. Brachial plexus birth palsy: management during the first year of life. Orthop Traumatol Surg Res. 2016;102(1 Suppl):S125-S132.
- Belzberg AJ, Dorsi MJ, Storm PB, Moriarity JL. Surgical repair of brachial plexus injury: a multinational survey of experienced peripheral nerve surgeons. J Neurosurg. 2004;101(3):365-376.
- Vanderhave KL, Bovid K, Alpert H, et al. Utility of electrodiagnostic testing and computed tomography myelography in the preoperative evaluation of neonatal brachial plexus palsy. J Neurosurg Pediatr.2012;9(3):283-289.
- Terzis JK, Karypidis D. Outcomes of direct muscle neurotization in pediatric patients with facial paralysis. Plast Reconstr Surg. 2009; 124(5):1486-1498.
- Kang PB. Approach to electrodiagnostic testing in children. In: McMillan HJ, Kang PB, eds. Pediatric Electromyography: Concepts and Clinical Applications. Cham, Switzerland: Springer; 2017:23-27.
- Pitt M. Paediatric Electromyography. Oxford: Oxford University Press; 2018. 352 p.
- Alshaikh NM, Martinez JP, Pitt MC. Perception of pain during electromyography in children: a prospective study. Muscle Nerve. 2016;54(3):422-426.
- McHugh JC. Sensory studies. In: McMillan HJ, Kang PB, eds. Pediatric Electromyography: Concepts and Clinical Applications. Cham, Switzerland: Springer; 2017:31-48.
- Hays RM, Hackworth SR, Speltz ML, Weinstein P. Exploration of variables related to children's behavioral distress during electrodiagnosis. Arch Phys Med Rehabil. 1992;73(12):1160-1162.
- Ballard A, Khadra C, Adler S, Trottier ED, Le May S. Efficacy of the buzzy device for pain management during needle-related procedures: a systematic review and meta-analysis. Clin J Pain. 2019; 35(6):532-543.
- Pitt MC. Nerve conduction studies and needle EMG in very small children. Eur J Paediatr Neurol. 2012;16(3):285-291.
- Thomas JE, Lambert EH. Ulnar nerve conduction velocity and Hreflex in infants and children. J Appl Physiol. 1960;15:1-9.
- Wagner AL, Buchthal F. Motor and sensory conduction in infancy and childhood: reappraisal. Dev Med Child Neurol. 1972;14(2): 189-216.
- Chen S, Andary M, Buschbacher R, et al. Electrodiagnostic reference values for upper and lower limb nerve conduction studies in adult populations. Muscle Nerve. 2016;54(3):371-377.
- Miller RG, Kuntz NL. Nerve conduction studies in infants and children. J Child Neurol. 1986;1(1):19-26.
- Lori S, Bertini G, Bastianelli M, et al. Peripheral nervous system maturation in preterm infants: longitudinal motor and sensory nerve conduction studies. Childs Nerv Syst. 2018;34(6):1145-1152.
- Dillingham T, Chen S, Andary M, et al. Establishing high-quality reference values for nerve conduction studies: a report from the normative data task force of the American Association of Neuromuscular & Electrodiagnostic Medicine. Muscle Nerve. 2016;54(3): 366-370.
- Smit BJ, Kok JH, De Vries LS, Dekker FW. Ongerboer de Visser BW. Motor nerve conduction velocity in very preterm infants. Muscle Nerve. 1999;22(3):372-377.
- Ryan CS, Conlee EM, Sharma R, Sorenson EJ, Boon AJ, Laughlin RS. Nerve conduction normal values for electrodiagnosis in pediatric patients. Muscle Nerve. 2019;60(2):155-160.
- Wang L, Hu Y, Jiang L, Zhong X, Chen J, Xu N. Electrophysiological characteristics of the pediatric femoral nerve and their use in clinical diagnosis. Pediatr Neurol. 2014;50(2):149-157.
- Cai F, Zhang J. Study of nerve conduction and late responses in normal Chinese infants, children, and adults. J Child Neurol. 1997;12(1): 13-18.
- Hyllienmark L, Ludvigsson J, Brismar T. Normal values of nerve conduction in children and adolescents. Electroencephalogr Clin Neurophysiol. 1995;97(5):208-214.
- Parano E, Uncini A, De Vivo DC, Lovelace RE. Electrophysiologic correlates of peripheral nervous system maturation in infancy and childhood. J Child Neurol. 1993;8(4):336-338.
- Lang HA, Puusa A, Hynninen P, Kuusela V, Jantti V, Sillanpaa M. Evolution of nerve conduction velocity in later childhood and adolescence. Muscle Nerve. 1985;8(1):38-43.
- Garcia A, Calleja J, Antolin FM, Berciano J. Peripheral motor and sensory nerve conduction studies in normal infants and children. Clin Neurophysiol. 2000;111(3):513-520.
- Jabre JF, Pitt MC, Deeb J, Chui KK. E-norms: a method to extrapolate reference values from a laboratory population. J Clin Neurophysiol. 2015;32(3):265-270.
- Dioszeghy P, Stalberg E. Changes in motor and sensory nerve conduction parameters with temperature in normal and diseased nerve. Electroencephalogr Clin Neurophysiol. 1992;85(4):229-235.
- Puksa L, Eeg-Olofsson KE, Stalberg E, Falck B. Reference values for F wave parameters in healthy 3-20 year old subjects. Clin Neurophysiol. 2011;122(1):199-204.
- Sharma R, Sorenson EJ, Ryan CS, Conlee EM, Boon AJ, Laughlin RS. Establishing normal values for F-Wave latencies in diagnosing peripheral neuropathies for the pediatric population. J Clin Neurophysiol. 2019. https://doi.org/10.1097/WNP. 0000000000000000609 [Epub ahead of print].
- Nadeem AS, El-Yassin DI, Al-Ani F. Analysis of F-wave parameters in normal infants and children. Ann Saudi Med. 2002;22(3–4):181-185.
- Misra UK, Tiwari S, Shukla N, Nishith SD, Malik GK, Nag D. Fresponse studies in neonates, infants and children. Electromyogr Clin Neurophysiol. 1989;29(4):251-254.
- Mayer RF, Mosser RS. Excitability of motoneurons in infants. Neurology. 1969;19(10):932-945.
- Pereon Y. Nguyen The Tich S, Fournier E, Genet R, Guiheneuc P. Electrophysiological recording of deep tendon reflexes: normative data in children and in adults. Neurophysiol Clin. 2004;34(3–4): 131-139.
- Mongiovi PC, Elsheikh B, Lawson VH, Kissel JT, Arnold WD. Neuromuscular junction disorders mimicking myopathy. Muscle Nerve. 2014;50(5):854-856.
- Kinali M, Beeson D, Pitt MC, et al. Congenital myasthenic syndromes in childhood: diagnostic and management challenges. J Neuroimmunol. 2008;201–202:6–12.
- Nicolau S, Kao JC, Liewluck T. Trouble at the junction: when myopathy and myasthenia overlap. Muscle Nerve. 2019;60(6):648–657.
- Kumar RS, Kuruvilla A. Repetitive compound muscle action potentials in electrophysiological diagnosis of congenital myasthenic syndromes: a case report and review of literature. Ann Indian Acad Neurol. 2010;13(2):139-141.
- van Dijk JG, Lammers GJ, Wintzen AR, Molenaar PC. Repetitive CMAPs: mechanisms of neural and synaptic genesis. Muscle Nerve. 1996;19(9):1127-1133.
- Ashraf VV, Taly AB, Veerendrakumar M, Rao S. Myasthenia gravis in children: a longitudinal study. Acta Neurol Scand. 2006;114(2): 119-123.
- Snead OC III, Benton JW, Dwyer D, et al. Juvenile myasthenia gravis. Neurology. 1980;30(7 Pt 1):732-739.
- Batocchi AP, Evoli A, Palmisani MT, Lo Monaco M, Bartoccioni M, Tonali P. Early-onset myasthenia gravis: clinical characteristics and response to therapy. Eur J Pediatr. 1990;150(1):66-68.
- Gilchrist JM, Massey JM, Sanders DB. Single fiber EMG and repetitive stimulation of the same muscle in myasthenia gravis. Muscle Nerve. 1994;17(2):171-175.
- Sanders DB, Howard JF Jr, Johns TR. Single-fiber electromyography in myasthenia gravis. Neurology. 1979;29(1):68-76.
- Pitt M. Neurophysiological assessment of abnormalities of the neuromuscular junction in children. Int J Mol Sci. 2018;19(2).
- Guan YZ, Cui LY, Liu MS, Niu JW. Single-fiber electromyography in the extensor digitorum communis for the predictive prognosis of ocular myasthenia gravis: a retrospective study of 102 cases. Chin Med J (Engl). 2015;128(20):2783-2786.
- Hays RM, Michaud LJ. Neonatal myasthenia gravis: specific advantages of repetitive stimulation over edrophonium testing. Pediatr Neurol. 1988;4(4):245-247.
- Dilena R, Abicht A, Sergi P, et al. Congenital myasthenic syndrome due to choline acetyltransferase mutations in infants: clinical suspicion and comprehensive electrophysiological assessment are important for early diagnosis. J Child Neurol. 2014;29(3):389-393.
- Gurnett CA, Bodnar JA, Neil J, Connolly AM. Congenital myasthenic syndrome: presentation, electrodiagnosis, and muscle biopsy. J Child Neurol. 2004;19(3):175-182.
- Zafeiriou DI, Pitt M, de Sousa C. Clinical and neurophysiological characteristics of congenital myasthenic syndromes presenting in early infancy. Brain Dev. 2004;26(1):47-52.
- Morgan-Followell B, de Los Reyes E. Child neurology: diagnosis of Lambert-Eaton myasthenic syndrome in children. Neurology. 2013; 80(21):e220-e222.
- Fournier E, Arzel M, Sternberg D, et al. Electromyography guides toward subgroups of mutations in muscle channelopathies. Ann Neurol. 2004;56(5):650-661.
- Michel P, Sternberg D, Jeannet PY, et al. Comparative efficacy of repetitive nerve stimulation, exercise, and cold in differentiating myotonic disorders. Muscle Nerve. 2007;36(5):643-650.
- Renault F. Pediatric cranial neuropathies. In: McMillan HJ, Kang PB, eds. Pediatric Electromyography: Concepts and Clinical Applications.
- Cham, Switzerland: Springer; 2017:181-198. Renault F. Facial electromyography in newborn and young infants with congenital facial weakness. Dev Med Child Neurol. 2001;43(6): 421-427.
- Vecchierini-Blineau MF, Guiheneuc P. Maturation of the blink reflex in infants. Eur Neurol. 1984;23(6):449-458.
- Blank A, Ferber I, Shapira Y, Fast A. Electrically elicited blink reflex in children. Arch Phys Med Rehabil. 1983;64(11):558-559.
- Tomita Y, Shichida K, Takeshita K, Takashima S. Maturation of blink reflex in children. Brain Dev. 1989;11(6):389-393.
- Vucic S, Cairns KD, Black KR, Chong PS, Cros D. Neurophysiologic findings in early acute inflammatory demyelinating polyradiculoneuropathy. Clin Neurophysiol. 2004;115(10):2329-2335.
- Koehler J, Schwarz M, Urban PP, Voth D, Holker C, Hopf HC. Masseter reflex and blink reflex abnormalities in Chiari II malformation. Muscle Nerve. 2001;24(3):425-427.
- Raffaele R, Rampello L, Vecchio I, et al. Blink reflex abnormalities in children with Tourette syndrome. Eur J Neurol. 2006;13(8):869-873.
- Anday EK, Cohen ME, Hoffman HS. The blink reflex: maturation and modification in the neonate. Dev Med Child Neurol. 1990;32(2): 142-150.
- Snir M, Axer-Siegel R, Bourla D, Kremer I, Benjamini Y, Weinberger D. Tactile corneal reflex development in full-term babies. Ophthalmology. 2002;109(3):526-529.
- Heath JP, Cull RE, Smith IM, Murray JA. The neurophysiological investigation of Bell's palsy and the predictive value of the blink reflex. Clin Otolaryngol Allied Sci. 1988;13(2):85-92.
- Dumitru D, Walsh NE, Porter LD. Electrophysiologic evaluation of the facial nerve in Bell's palsy. A review. Am J Phys Med Rehabil. 1988;67(4):137-144.
- Wong V. Outcome of facial nerve palsy in 24 children. Brain Dev. 1995;17(4):294-296.
- Jaradeh S, D'Cruz O, Howard JF Jr, Haberkamp TJ, Konkol RJ. Mobius syndrome: electrophysiologic studies in seven cases. Muscle Nerve. 1996;19(9):1148-1153.
- Hatanaka T, Yoshijima S, Hayashi N, Owa K, Suehiro Y, Shinomiya K. Electrophysiologic studies in an infant with Mobius syndrome. J Child Neurol. 1993;8(2):182-185.
- Orhan EK, Kirac LB, Dikmen PY, et al. Electromyography in Pediatric Population. Noro Psikiyatr Ars. 2018;55(1):36-39.
- Hellmann M, von Kleist-Retzow JC, Haupt WF, Herkenrath P, Schauseil-Zipf U. Diagnostic value of electromyography in children and adolescents. J Clin Neurophysiol. 2005;22(1):43-48.
- Ghosh PS, Sorenson EJ. Diagnostic yield of electromyography in children with myopathic disorders. Pediatr Neurol. 2014;51(2):215-219.
- Rabie M, Jossiphov J, Nevo Y. Electromyography (EMG) accuracy compared to muscle biopsy in childhood. J Child Neurol. 2007;22(7): 803-808.
- Brownell AA, Bromberg MB. Comparison of standard and pediatric size concentric needle EMG electrodes. Clin Neurophysiol. 2007; 118(5):1162-1165.
- Zaidman CM, van Alfen N. Ultrasound in the assessment of myopathic disorders. J Clin Neurophysiol. 2016;33(2):103-111.
- Racca F, Mongini T, Wolfler A, et al. Recommendations for anesthesia and perioperative management of patients with neuromuscular disorders. Minerva Anestesiol. 2013;79(4):419-433.
- Massey JM. Electromyography in disorders of neuromuscular transmission. Semin Neurol. 1990;10(1):6-11.
- Bhatia S, Quinlan H, McCracken C, Price EW, Guglani L, Verma S. Serial stimulated jitter analysis in juvenile myasthenia gravis. Muscle Nerve. 2018;58(5):729-732.
- Pitt MC. Use of stimulated electromyography in the analysis of the neuromuscular junction in children. Muscle Nerve. 2017;56(5): 841-847.
- Pitt MC, McHugh JC, Deeb J, Smith RA. Assessing neuromuscular junction stability from stimulated EMG in children. Clin Neurophysiol. 2017;128(2):290-296.
- Tidswell T, Pitt MC. A new analytical method to diagnose congenital myasthenia with stimulated single-fiber electromyography. Muscle Nerve. 2007;35(1):107-110.
- Verma S, Lin J. Stimulated jitter analysis for the evaluation of neuromuscular junction disorders in children. Muscle Nerve. 2016;53(3): 471-472.
- Sanders DB, Arimura K, Cui L, et al. Guidelines for single fiber EMG. Clin Neurophysiol. 2019;130(8):1417-1439.
- Stalberg E, Kouyoumdjian JA, Paiva GP, Yanaze LL, Sanders DB. Problems in comparing jitter values obtained with voluntary activation and electrical stimulation. J Neuromuscul Dis. 2018;5(2): 225-230.
- Pitt MC, Jabre JF. Determining jitter values in the very young by use of the e-norms methodology. Muscle Nerve. 2017;55(1):51-54.
- Cook-Norris RH, Tollefson MM, Cruz-Inigo AE, Sandroni P, Davis MD, Davis DM. Pediatric erythromelalgia: a retrospective review of 32 cases evaluated at Mayo Clinic over a 37-year period. J Am Acad Dermatol. 2012;66(3):416-423.
- Biegstraaten M, Binder A, Maag R, Hollak CE, Baron R, van Schaik IN. The relation between small nerve fibre function, age, disease severity and pain in Fabry disease. Eur J Pain. 2011;15(8): 822-829.
- Hoeijmakers JG, Faber CG, Miedema CJ, Merkies IS, Vles JS. Small fiber neuropathy in children: two case reports illustrating the importance of recognition. Pediatrics. 2016;138(4).
- Oaklander AL, Klein MM. Evidence of small-fiber polyneuropathy in unexplained, juvenile-onset, widespread pain syndromes. Pediatrics. 2013;131(4):e1091-e1100.
- Kafaie J, Al Balushi A, Kim M, Pestronk A. Clinical and laboratory profiles of idiopathic small fiber neuropathy in children: case series. J Clin Neuromuscul Dis. 2017;19(1):31-37.
- Hilz MJ, Axelrod FB. Quantitative sensory testing of thermal and vibratory perception in familial dysautonomia. Clin Auton Res. 2000; 10(4):177-183.
- Kuntz NL. Diagnosis and evaluation of small fiber peripheral neuropathy in children. In: McMillan HJ, Kang PB, eds. Pediatric Electromyography: Concepts and Clinical Applications. Cham, Switzerland: Springer; 2017:265-280.
- Shahani BT, Halperin JJ, Boulu P, Cohen J. Sympathetic skin response– a method of assessing unmyelinated axon dysfunction in peripheral neuropathies. J Neurol Neurosurg Psychiatry. 1984;47(5):536-542.
- Treister R, Lodahl M, Lang M, Tworoger SS, Sawilowsky S, Oaklander AL. Initial development and validation of a patient-reported symptom survey for small-fiber polyneuropathy. J Pain. 2017;18(5): 556-563.
- Meier PM, Berde CB, DiCanzio J, Zurakowski D, Sethna NF. Quantitative assessment of cutaneous thermal and vibration sensation and thermal pain detection thresholds in healthy children and adolescents. Muscle Nerve. 2001;24(10):1339-1345.
- Loseth S, Lindal S, Stalberg E, Mellgren SI. Intraepidermal nerve fibre density, quantitative sensory testing and nerve conduction studies in a patient material with symptoms and signs of sensory polyneuropathy. Eur J Neurol. 2006;13(2):105-111.
- Panoutsopoulou IG, Luciano CA, Wendelschafer-Crabb G, Hodges JS, Kennedy WR. Epidermal innervation in healthy children and adolescents. Muscle Nerve. 2015;51(3):378-384.
- Schneider C, Bucher F, Cursiefen C, Fink GR, Heindl LM, Lehmann HC. Corneal confocal microscopy detects small fiber damage in chronic inflammatory demyelinating polyneuropathy (CIDP). J Peripher Nerv Syst. 2014;19(4):322-327.
- Lagerburg V, Bakkers M, Bouwhuis A, et al. Contact heat evoked potentials: normal values and use in small-fiber neuropathy. Muscle Nerve. 2015;51(5):743-749.
- Fealey RD. Thermoregulatory sweat test. In: Low PA, ed. Clinical Autonomic Disorders. 3rd ed. Baltimore, MD: Lippincott Williams & Wilkins; 2008:244-263.
- Kuntz NL, Patwari PP. Laboratory evaluation of pediatric autonomic disorders. Semin Pediatr Neurol. 2013;20(1):35-43.
- Fagan ER, Taylor MJ, Logan WJ. Somatosensory evoked potentials: part II. A review of the clinical applications in pediatric neurology. Pediatr Neurol. 1987;3(5):249-255.
- Azabou E, Manel V, Andre-obadia N, et al. Optimal parameters of transcranial electrical stimulation for intraoperative monitoring of motor evoked potentials of the tibialis anterior muscle during pediatric scoliosis surgery. Neurophysiol Clin. 2013;43(4):243-250.
- Holdefer RN, Anderson C, Furman M, Sangare Y, Slimp JC. A comparison of the effects of desflurane versus propofol on transcranial motor-evoked potentials in pediatric patients. Childs Nerv Syst. 2014;30(12):2103-2108.
- Mahmoud M, Sadhasivam S, Sestokas AK, Samuels P, McAuliffe J. Loss of transcranial electric motor evoked potentials during pediatric spine surgery with dexmedetomidine. Anesthesiology. 2007;106(2): 393-396.
Document History
Creation of New Guidelines, Consensus Statements, or Position Papers
AANEM members are encouraged to submit ideas for papers that can improve the understanding of the field. The AANEM will review nominated topics on the basis of the following criteria:- Members’ needs
- Prevalence of condition
- Health impact of condition for the individual and others
- Socioeconomic impact
- Extent of practice variation
- Quality of available evidence
- External constraints on practice
- Urgency for evaluation of new practice technology